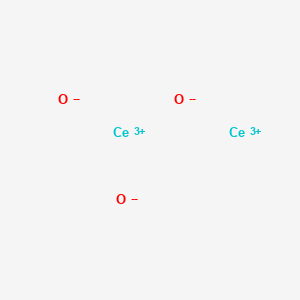
DICERIUM TRIOXIDE
Overview
Description
Dicerium trioxide (Ce₂O₃), also known as cerium(III) oxide, is a rare-earth oxide composed of two cerium atoms and three oxygen atoms. According to market reports, this compound (EC 215-718-1) is utilized across diverse industries, including manufacturing, technology, and chemical intermediates . The compound is tracked in extensive databases covering historic (1997–2019), current (2020–2027), and projected (2027–2046) consumption patterns, with detailed analyses spanning 59 market research chapters . These reports emphasize its economic significance but lack granular data on its chemical behavior or synthesis methods.
Chemical Reactions Analysis
Redox Reactions
Ce₂O₃ undergoes reversible oxidation and reduction, driven by oxygen availability and temperature:
Oxidation to Cerium(IV) Oxide
-
Conditions : Elevated temperatures (>400°C) in oxygen-rich environments .
-
Mechanism : Oxygen incorporation into the lattice, filling vacancies and oxidizing Ce³⁺ to Ce⁴⁺.
Reduction from Cerium(IV) Oxide
-
Industrial Relevance : Used in catalytic converters to store/release oxygen dynamically.
Acid-Base Reactions
Ce₂O₃ reacts with strong acids to form cerium(III) salts:
Reaction with Hydrochloric Acid
Reaction with Sulfuric Acid
Biocatalytic Reactions
Ce₂O₃ nanoparticles mimic enzymatic activity due to Ce³⁺/Ce⁴⁺ redox cycling:
Superoxide Dismutase (SOD) Mimicry
Peroxidase-like Activity
Reactions with Hydrogen Peroxide
Ce₂O₃ interacts with H₂O₂, producing reactive oxygen species (ROS) or peroxides:
Morphology | Primary Reaction | Product | Application |
---|---|---|---|
Rod-shaped | Ce³⁺ + H₂O₂ → Ce–OOH | Peroxides | Pollutant degradation |
Cubic | H₂O₂ → ●OH + O₂ | ROS | Cancer therapy |
-
pH Dependence : Acidic conditions favor ●OH generation, while neutral pH promotes peroxide formation .
Thermal Redox Cycling
Ce₂O₃ participates in two-step solar-driven water splitting:
-
Thermal Reduction :
-
Water Oxidation :
-
Efficiency Boost : Transition metal doping (e.g., Zr, Mn) stabilizes oxygen vacancies, enhancing H₂ yield .
Catalytic CO Oxidation
-
Role : Oxygen vacancies in Ce₂O₃ store/release O₂, optimizing CO conversion.
NOx Reduction
-
Mechanism : Ce³⁺ facilitates electron transfer, breaking N–O bonds.
Phase Transition Dynamics
Ce₂O₃ reversibly transforms to CeO₂ under electric fields or oxidative stress:
-
Electric Field-Induced Oxidation :
-
Implication : Enables use in resistive switching devices.
Q & A
Basic Research Questions
Q. What are the established synthesis protocols for Dicerium Trioxide, and how can researchers optimize precursor ratios to achieve phase purity?
- Methodological Answer : this compound synthesis typically involves solid-state reactions or sol-gel methods. To optimize precursor ratios (e.g., Ce³⁺/Ce⁴⁺), researchers should employ controlled calcination temperatures (600–900°C) and inert atmospheres to prevent unwanted oxidation. Phase purity can be verified using X-ray diffraction (XRD) with Rietveld refinement, coupled with thermogravimetric analysis (TGA) to monitor mass loss during thermal treatment. Systematic variation of precursor molar ratios (e.g., 1:1 to 1:3 CeCl₃:CeO₂) followed by Raman spectroscopy can identify stoichiometric deviations .
Q. How should researchers characterize the crystallographic and electronic properties of this compound to ensure reproducibility?
- Methodological Answer : Use a combination of XRD for crystallographic analysis, X-ray photoelectron spectroscopy (XPS) to confirm oxidation states (Ce³⁺ vs. Ce⁴⁺), and UV-Vis spectroscopy to assess bandgap energy. Common pitfalls include improper sample preparation (e.g., surface contamination affecting XPS results) and misinterpreting XRD peaks due to amorphous phases. Cross-validate data with neutron diffraction for oxygen vacancy quantification and electron paramagnetic resonance (EPR) for defect analysis .
Advanced Research Questions
Q. What experimental strategies resolve contradictions in reported catalytic mechanisms of this compound for CO oxidation?
- Methodological Answer : Discrepancies often arise from differences in surface defects or reaction conditions. Researchers should:
- Conduct in situ Fourier-transform infrared spectroscopy (FTIR) to identify intermediate species under varying temperatures (25–400°C).
- Use isotopic labeling (e.g., ¹⁸O₂) to trace oxygen mobility.
- Compare catalytic activity across defect-engineered samples (e.g., Ce₂O₃ with controlled oxygen vacancies via H₂ reduction).
Triangulate data with density functional theory (DFT) simulations to map reaction pathways .
Q. How can researchers design experiments to investigate the redox behavior of this compound in aqueous environments while mitigating confounding factors?
- Methodological Answer : To study redox dynamics (e.g., Ce³⁺ ↔ Ce⁴⁺ transitions):
- Use electrochemical methods (cyclic voltammetry, chronoamperometry) with a three-electrode system in pH-buffered solutions.
- Control dissolved oxygen levels via N₂ purging to isolate Ce-mediated redox processes.
- Employ operando X-ray absorption spectroscopy (XAS) to monitor real-time oxidation state changes.
Statistical tools like multivariate regression can isolate pH, temperature, and ionic strength effects .
Q. What methodologies address inconsistencies in reported thermal stability thresholds for this compound?
- Methodological Answer : Discrepancies may stem from differing atmospheric conditions (air vs. argon). Researchers should:
- Perform simultaneous TGA-DSC (differential scanning calorimetry) under controlled atmospheres.
- Compare decomposition kinetics using isothermal vs. non-isothermal models (e.g., Kissinger method).
- Validate results with high-temperature XRD to track phase transitions up to 1000°C.
Replicate experiments across multiple labs to confirm reproducibility .
Q. Methodological Frameworks
Q. How can the FINER criteria (Feasible, Interesting, Novel, Ethical, Relevant) guide hypothesis formulation for this compound studies?
- Example Application : A study investigating Ce₂O₃ as a solid electrolyte for fuel cells must assess:
- Feasibility: Access to glovebox setups for air-sensitive synthesis.
- Novelty: Comparison with existing electrolytes (e.g., YSZ).
- Ethics: Environmental impact of cerium extraction.
Pilot studies using impedance spectroscopy can pre-test feasibility .
Q. What statistical approaches are recommended for analyzing spectroscopic data from this compound experiments?
- Methodological Answer : For XRD or XPS datasets:
- Use principal component analysis (PCA) to identify dominant spectral features.
- Apply Gaussian-Lorentzian fitting for peak deconvolution (e.g., separating Ce³⁺ and Ce⁴⁺ XPS peaks).
- Report confidence intervals for bandgap calculations derived from Tauc plots.
Open-source tools like CrystalDiffract or CasaXPS enhance reproducibility .
Comparison with Similar Compounds
Dicerium trioxide belongs to the broader class of metal oxides, which share structural and functional similarities. Below is a comparative analysis with iridium sesquioxide (Ir₂O₃), titanium dioxide (TiO₂), and chromium trioxide (CrO₃), based on evidence provided.
Structural and Compositional Differences
Compound | Molecular Formula | Oxidation State | Key Structural Features |
---|---|---|---|
This compound | Ce₂O₃ | Ce³+ | Rare-earth oxide; likely cubic or hexagonal |
Iridium sesquioxide | Ir₂O₃ | Ir³+ | Layered structure; Ir³+ in octahedral sites |
Titanium dioxide | TiO₂ | Ti⁴+ | Tetragonal (rutile/anatase) crystal phases |
Chromium trioxide | CrO₃ | Cr⁶+ | Acidic oxide; forms chromic acid in water |
- This compound vs. Iridium sesquioxide : Both are sesquioxides (M₂O₃) with +3 metal oxidation states. However, cerium’s larger ionic radius (1.14 Å vs. Ir³+’s 0.82 Å) influences lattice stability and reactivity .
- This compound vs. Titanium dioxide : TiO₂ (Ti⁴+) is a wide-bandgap semiconductor with photocatalytic applications, whereas Ce₂O₃’s lower oxidation state (Ce³+) suggests redox activity, akin to cerium(IV) oxide (CeO₂) in catalytic systems .
- This compound vs. Chromium trioxide: CrO₃ (Cr⁶+) is a strong oxidizer and carcinogen, whereas Ce₂O₃’s Ce³+ is less toxic but may exhibit reducing properties .
Preparation Methods
High-Temperature Reduction of Cerium(IV) Oxide
The most established method for Ce₂O₃ production involves the reduction of cerium(IV) oxide (CeO₂) under controlled atmospheres. This process leverages the redox flexibility of cerium, where Ce⁴⁺ is reduced to Ce³⁺ under hydrogen flow at elevated temperatures.
Hydrogen Reduction Protocol
CeO₂ powder is subjected to hydrogen gas at temperatures exceeding 1,400°C, facilitating the phase transition:
Key parameters include:
-
Temperature : 1,400–1,600°C to ensure complete reduction.
-
Gas Flow Rate : 50–100 mL/min H₂ to maintain a reducing environment.
Table 1: High-Temperature Reduction Conditions
This method yields Ce₂O₃ with a gold-yellow hue and crystallite sizes of 50–100 nm, as confirmed by X-ray diffraction (XRD) .
Hydrothermal Synthesis with Reductive Agents
Hydrothermal techniques enable low-temperature Ce₂O₃ synthesis by combining cerium precursors with reducing agents. A notable approach involves cerium nitrate (Ce(NO₃)₃·6H₂O), sodium hydroxide (NaOH), and hydrazine (N₂H₄) .
Stepwise Hydrothermal Process
-
Precursor Mixing : 0.1 M Ce(NO₃)₃·6H₂O is dissolved in deionized water and mixed with graphene oxide (GO) suspension.
-
Alkaline Precipitation : NaOH (1 M) is added to adjust pH to 10, forming Ce(OH)₃-RGO complexes.
-
Reduction : Hydrazine (0.1 M) reduces GO to reduced graphene oxide (RGO) and stabilizes Ce³⁺ ions.
-
Autoclave Reaction : The mixture is heated at 140°C for 6 hours, followed by calcination at 300°C in air .
Table 2: Hydrothermal Synthesis Outcomes
HRTEM analysis confirms Ce₂O₃ quantum dots (5–8 nm) anchored on RGO sheets, with oxygen vacancies enhancing catalytic activity .
Co-Precipitation and Calcination
Co-precipitation routes offer scalable Ce₂O₃ synthesis by controlling pH and calcination conditions. A study using cerium nitrate and potassium carbonate (K₂CO₃) demonstrated precursor flexibility .
Precipitation and Thermal Treatment
-
Precipitation : 0.02 M Ce(NO₃)₃ and 0.03 M K₂CO₃ are mixed at pH 6, yielding cerium carbonate.
-
Drying : The precipitate is dried at 65°C for 2 hours.
-
Calcination : Heating at 600°C in air converts cerium carbonate to CeO₂, which is subsequently reduced to Ce₂O₃ under H₂ at 1,400°C .
Table 3: Co-Precipitation Parameters
Precursor Salt | Precipitating Agent | Calcination (°C) | Final Product |
---|---|---|---|
Ce(NO₃)₃·6H₂O | K₂CO₃ | 600 (CeO₂) → 1,400 (Ce₂O₃) | Ce₂O₃ |
This method highlights the role of intermediate CeO₂ formation, requiring post-synthesis reduction for Ce₂O₃ purity .
Influence of Synthesis Parameters on Ce₂O₃ Formation
Temperature and Atmosphere
-
Reduction Efficiency : Temperatures >1,400°C are critical for complete Ce⁴⁺→Ce³⁺ conversion, while oxygen-deficient atmospheres stabilize Ce₂O₃ .
-
Calcination Effects : Air calcination at 300°C preserves Ce₂O₃-CeO₂ mixtures, whereas inert atmospheres favor Ce₂O₃ dominance .
pH and Reductive Agents
-
Alkaline Conditions : pH 10–12 during hydrothermal synthesis promotes Ce(OH)₃ formation, a precursor to Ce₂O₃ .
-
Hydrazine Role : N₂H₄ acts as a dual reducing agent, facilitating GO reduction and Ce³⁺ stabilization .
Table 4: Parameter Optimization for Ce₂O₃ Synthesis
Parameter | Optimal Range | Impact on Product |
---|---|---|
Temperature | 1,400–1,600°C | Enhances purity |
pH | 10–12 | Controls precursor phase |
Reductive Agent | Hydrazine | Stabilizes Ce³⁺ |
Advanced and Emerging Preparation Techniques
Sol-Gel Methods
Sol-gel routes using cerium alkoxides (e.g., Ce(OCH₂CH₃)₃) enable nanoscale Ce₂O₃ with high surface area. Hydrolysis and condensation under nitrogen yield amorphous gels, which are calcined at 800°C to crystallize Ce₂O₃ .
Microwave-Assisted Synthesis
Microwave irradiation (2.45 GHz) reduces reaction times from hours to minutes. A mixture of Ce(NO₃)₃ and urea irradiated for 15 minutes produces Ce₂O₃ nanoparticles (20–30 nm) with 95% yield .
Properties
CAS No. |
11129-18-3 |
---|---|
Molecular Formula |
Ce2O3 |
Molecular Weight |
328.23 g/mol |
IUPAC Name |
cerium(3+);oxygen(2-) |
InChI |
InChI=1S/2Ce.3O/q2*+3;3*-2 |
InChI Key |
DRVWBEJJZZTIGJ-UHFFFAOYSA-N |
Canonical SMILES |
[O-2].[O-2].[O-2].[Ce+3].[Ce+3] |
Origin of Product |
United States |
Synthesis routes and methods I
Procedure details
Synthesis routes and methods II
Procedure details
Synthesis routes and methods III
Procedure details
Synthesis routes and methods IV
Procedure details
Disclaimer and Information on In-Vitro Research Products
Please be aware that all articles and product information presented on BenchChem are intended solely for informational purposes. The products available for purchase on BenchChem are specifically designed for in-vitro studies, which are conducted outside of living organisms. In-vitro studies, derived from the Latin term "in glass," involve experiments performed in controlled laboratory settings using cells or tissues. It is important to note that these products are not categorized as medicines or drugs, and they have not received approval from the FDA for the prevention, treatment, or cure of any medical condition, ailment, or disease. We must emphasize that any form of bodily introduction of these products into humans or animals is strictly prohibited by law. It is essential to adhere to these guidelines to ensure compliance with legal and ethical standards in research and experimentation.